Difference between revisions of "Radiation shielding"
Line 173: | Line 173: | ||
|- | |- | ||
|Boron nitride nanotubes | |Boron nitride nanotubes | ||
− | |Low atomic numbers; | + | |Low atomic numbers; boron absorbs secondary neutrons well compared to other elements; possible use as both shielding and structural material<ref>Tiano, Amanda L, et al. “Boron Nitride Nanotube: Synthesis and Applications.” NTRS Document ID 20140004051, 2014. <nowiki>http://hdl.handle.net/2060/20140004051</nowiki></ref>; hydrogen could be stored in or bonded to nanotubes to improve shielding<ref>Thibeault SA, Fay CC, Lowther SE, Earle KD, Sauti G, Kang JH, Park C, McMullen AM. (2012). ''Radiation Shielding Materials Containing Hydrogen, Boron, and Nitrogen: Systematic Computational and Experimental Study. Phase I''. <nowiki>https://ntrs.nasa.gov/search.jsp?R=20160010096</nowiki></ref> |
|Difficult to manufacture | |Difficult to manufacture | ||
|} | |} |
Revision as of 15:14, 12 May 2020
Shielding against radiation is considered a very difficult task. For example, a proton or alpha particle cosmic ray of "medium" energy can pass through more than a meter of aluminium, not counting the effects of secondary radiation[1]. With this in mind, it is clear that any Martian colonists would have to take a holistic approach, reducing their radiation exposure at every possible opportunity through shielding and risk-mitigating behaviour.
Contents
Passive shielding
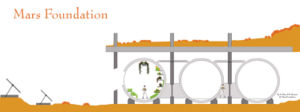
In most cases, matter placed between a person (or radiation-sensitive equipment) and radiation source reduces the amount of radiation they absorb.
Mars One's solution is a thick layer of regolith on top of the settlement modules. An effective shield will require at least several hundred grams of regolith per square centimeter, according to one study.[2] Using a regolith density estimate of 1.4 g/cm3[3], this means the regolith layer would need to be over 2 meters deep. For concrete with an average density of 2.4 g/cm3 the required thickness might be less.
Protection from Electromagnetic Radiation
The attenuation of radiation follows the Beer Lamberth law.[4]
Ix=Io*e-ux
Where: | I | = | the intensity of photons transmitted across some distance x |
I0 | = | the initial intensity of photons (or radiation in general) | |
s | = | a proportionality constant that reflects the total probability of a photon being scattered or absorbed | |
µ | = | the linear attenuation coefficient | |
x | = | distance traveled (thickness of material) |
Absorber | 100 keV | 200 keV | 500 keV |
---|---|---|---|
Air | 0.000195 | 0.000159 | 0.000112 |
Water | 0.167 | 0.136 | 0.097 |
Carbon | 0.335 | 0.274 | 0.196 |
Aluminium | 0.435 | 0.324 | 0.227 |
Iron | 2.72 | 1.09 | 0.655 |
Copper | 3.8 | 1.309 | 0.73 |
Lead | 59.7 | 10.15 | 1.64 |
the linear attenuation coefficient µ is not commonly found in the litterature, the mass attenuation coefficient µm is usually used instead. The coefficient is also dependent on the type of radiation, so a complete solution for radiation protection requires multiple analysis of the type of radiation to be protected against.
Conversion is quite simple as:
µ=µm*density of the material
List of mass attenuation coefficients[6] can be found at the NIST website. https://physics.nist.gov/PhysRefData/XrayMassCoef/tab3.html
Another common way of evaluating radiation shielding is to use the half value, that expresses the thickness of absorbing material which is needed to reduce the incident radiation intensity by a factor of two, or Ix=Io / 2.
The Half Value Layer for a range of absorbers is listed in the following table for three gamma-ray energies:
Absorber | 100 keV | 200 keV | 500 keV |
---|---|---|---|
Air | 3555 | 4359 | 6189 |
Water | 4.15 | 5.1 | 7.15 |
Carbon | 2.07 | 2.53 | 3.54 |
Aluminium | 1.59 | 2.14 | 3.05 |
Iron | 0.26 | 0.64 | 1.06 |
Copper | 0.18 | 0.53 | 0.95 |
Lead | 0.012 | 0.068 | 0.42 |
The first point to note is that the Half Value Layer decreases as the atomic number increases. For example, the value for air at 100 keV is about 35 meters and it decreases to just 0.12 mm for lead at this energy. In other words 35 m of air is needed to reduce the intensity of a 100 keV gamma-ray beam by a factor of two whereas just 0.12 mm of lead can do the same thing. The Half Value Layer increases with increasing gamma-ray energy. For example, from 0.18 cm for copper at 100 keV to about 1 cm at 500 keV.
Protection from Particulate Radiation
On Earth, particulate radiation is often easily addressed because the particles have low enough energies that they can be stopped by a thin shield. In space and on the surface of Mars, shielding needs to account for high-energy particles. When it comes to particulate radiation, the effectiveness of shielding increases with the mass of the shielding and decreases with the atomic mass of the elements used for the shielding. The reason that low-atomic-mass elements are advantageous is that they generate less secondary radiation when impacted by particles.[7] For example, 1kg of hydrogen offers more protection then 1kg of aluminium, 2kg of aluminium offers more protection than 1kg of aluminium and 1kg of hydrogen offers more protection than 2kg of aluminium.[8] Also, particles interact with atomic nuclei, while electromagnetic radiation interacts with electrons. So while for electromagnetic radiations the effectiveness of shielding increases with the number of electrons, and therefore with heavier atoms that have more electrons, for particles the effectiveness of radiation protection increases with the number of nuclei per volume, and lighter materials such as hydrogen have more nuclei per volume.
Possible Shielding Materials
Material | Advantages | Disadvantages |
---|---|---|
Metal | Efficiency of using structural material for incidental shielding benefit; some metals block EM radiation very well | Secondary radiation[9] |
Plastic | High hydrogen content[9] | Less structural utility than metal |
Water | High hydrogen content[9] | Liquid |
Liquid hydrogen | Pure hydrogen | Cryogenic liquid |
Regolith | Obtainable through ISRU | Large thickness required for thorough shielding[10] |
Regolith plus epoxy | Mostly obtainable through ISRU; greater hydrogen content than regolith alone; more durable and easier to shape than regolith alone[3] | More complex to implement than regolith alone |
Boron nitride nanotubes | Low atomic numbers; boron absorbs secondary neutrons well compared to other elements; possible use as both shielding and structural material[11]; hydrogen could be stored in or bonded to nanotubes to improve shielding[12] | Difficult to manufacture |
Active shielding
Active shielding against radiation involves a man made magnetic field which deflects ionized particles in the same manner as the Earth's. Such fields might require infeasible amounts of energy to generate and could also pose a major risk to anyone approaching the craft or base, as it would create bands of trapped particles similar to the Van Allen belts.[1] However, the radiation exposure might be low, as traversing the magnetic shield should be a very brief event.
It might be possible to situate a base in such a location that one of the residual Martian magnetic fields offers a net benefit. Care should certainly be taken not to situate it where the fields concentrate radiation.
Also, it might be possible (assuming one could generate the required magnetic field in some way) to have the radiation belts of the habitat pass through some sort of physical barrier, which scrubs them of particles.
Risk-mitigating behaviour
The possible sources of radiation on Mars are manmade sources, such as nuclear reactors or medical equipment, solar radiation, galactic cosmic radiation and naturally occuring radioactive elements on Mars.
Possible behavioural choices which minimize the risk from these include:
- Avoiding daytime EVA when there is a significant risk from solar radiation.
- Working preferentially close to natural or manmade objects, such as habitats, rovers or cliffs which provide additional (if not omni-directional) shielding.
- Entering a storm shelter when there is a high-radiation risk from solar particle events.
Example of using shielding and behavior to reduce radiation dosage
We can combine passive shielding with risk mitigating behavior to achieve low radiation exposure but still allow for some views of the exterior through windows. For example:
- Martian background average radiation is 240-300 mSv per year[13].
- If you sleep in a radiation shielded space such as underground rooms with a thick regolith cover, 8/24 hours, then the dose would go down by 1/3, to 160-200 mSv per year.
- If you spend most of your living (work, study) time in a radiation shielded space, then your dose becomes another 1/3 less, or 80 to 100 mSv.
- With overhangs and a radiation proof roof, 70% of the incident radiation to a space close to windows can be stopped by geometries, then the dose is down to 20 to 25 mSv. this is about the 20 mSv per year for a 5 year period that is recommended for radiation workers.
- Part of the surface dose on Mars is solar proton events. These are predictable and detectable, and a large settlement will mostly be built of shielded areas. So during Solar Proton Events you should stay away from the windows. This behavior might reduce the yearly radiation load another 25%, down to 15-18 mSv per year.
- What is the portion of the dosage from SPE? I have a weak reference that puts this at 30%. If correct, then the radiation load from large windows under a radiation proof ceiling is acceptable.
- Mars should be low in Radon because it is low in Thorium, and any thorium in the atmosphere can be separated out when the habitat atmosphere is produced. As 2 mSv on Earth comes from atmospheric radon, then this part of the yearly dose goes away.
- Even just 1/2 to 1 inches of glass reduces radiation dosage.
If the above is correct, then large windows are not really an issue. Geodesic glass domes over public spaces might be a poor choice, unless there is an understanding that you don't spend more than 2 to 4 hours per day under them.
References
- ↑ Jump up to: 1.0 1.1 Operational medicine and health care delivery - J.S. Logan, in S.E. Churchill ed. Fundamentals of space life sciences, Volume 1 - 1997, ISBN 0-89464-051-8 pp. 154-156.
- ↑ Slaba, T. C., Mertens, C. J., & Blattnig, S. R. (2013). Radiation Shielding Optimization on Mars. NASA/TP–2013-217983. Retrieved from https://ntrs.nasa.gov/archive/nasa/casi.ntrs.nasa.gov/20130012456.pdf
- ↑ Jump up to: 3.0 3.1 Kim, M. Y., Thibeault, S. A., Simonsen, L. C., & Wilson, J. W. Comparison of Martian Meteorites and Martian Regolith as Shield Materials for Galactic Cosmic Rays. NASA TP-1998-208724. Retrieved from https://ntrs.nasa.gov/archive/nasa/casi.ntrs.nasa.gov/19980237030.pdf.
- ↑ https://www.nde-ed.org/EducationResources/CommunityCollege/Radiography/Physics/attenuationCoef.htm
- ↑ Jump up to: 5.0 5.1 https://en.wikibooks.org/wiki/Basic_Physics_of_Nuclear_Medicine/Attenuation_of_Gamma-Rays
- ↑ https://www.nde-ed.org/EducationResources/CommunityCollege/Radiography/Physics/attenuationCoef.htm
- ↑ Wilson JW, Cucinotta FA, Thibeault SA, Kim M, Shinn JL, Badavi FF. Radiation Shielding Design Issues. In *Shielding Strategies for Human Space Exploration* (Chapter 7). http://hdl.handle.net/2060/19980137598
- ↑ Radiation biology - J.R. Letaw, in S.E. Churchill ed. Fundamentals of space life sciences, Volume 1 - 1997, ISBN 0-89464-051-8 pp. 16-17.
- ↑ Jump up to: 9.0 9.1 9.2 Parker LJ. (2016). Human radiation exposure tolerance and expected exposure during colonization of the Moon and Mars. http://www.marspapers.org/paper/Parker_2016_1.pdf
- ↑ James G, Chamitoff G, and Barker D. Resource Utilization and Site Selection for a Self-Sufficient Martian Outpost. NASA/TM-98-206538. http://hdl.handle.net/2060/19980147990
- ↑ Tiano, Amanda L, et al. “Boron Nitride Nanotube: Synthesis and Applications.” NTRS Document ID 20140004051, 2014. http://hdl.handle.net/2060/20140004051
- ↑ Thibeault SA, Fay CC, Lowther SE, Earle KD, Sauti G, Kang JH, Park C, McMullen AM. (2012). Radiation Shielding Materials Containing Hydrogen, Boron, and Nitrogen: Systematic Computational and Experimental Study. Phase I. https://ntrs.nasa.gov/search.jsp?R=20160010096
- ↑ NASA, Tony C. Slaba, Christopher J. Mertens, and Steve R. Blattnig Radiation Shielding Optimization on Mars , https://spaceradiation.larc.nasa.gov/nasapapers/NASA-TP-2013-217983.pdf, Apr 2013.